Bile salts and bile acids are polar cholesterol derivatives, and represent the major route for the elimination of the steroid from the body.
They are molecules with similar but not identical structures, and diverse physical and biological characteristics.
They are synthesized in the liver, stored in the gallbladder, secreted into the duodenum, and finally, for the most part, reabsorbed in the ileum.
Because at physiological pH these molecules are present as anions, the terms bile acid and bile salts are used herein as synonyms.
Contents
- Chemical structure
- Primary, conjugated and secondary bile salts
- Function of bile acids
- Enterohepatic circulation
- Synthesis
- References
Chemical structure
Bile salts have similarities and differences with cholesterol molecule.
Like the steroid, they have a nucleus composed of four fused rings: three cyclohexane rings, labeled A, B and C, and a cyclopentane ring, labeled D. This structure is the perhydrocyclopentanophenanthrene, more commonly known as steroid nucleus.
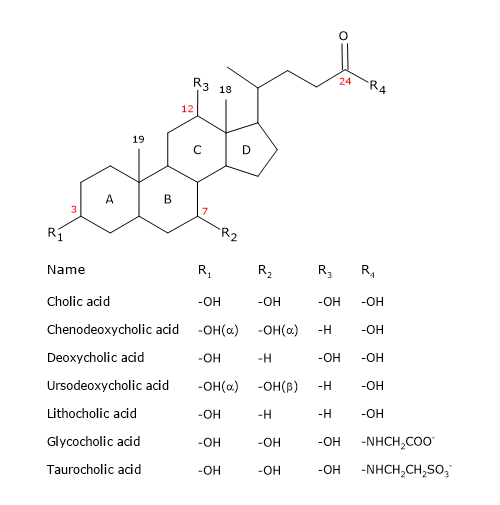
In higher vertebrates, they have 24 carbon atoms, as the side chain is three carbons shorter than the original. In lower vertebrates, bile acids have 25, 26, or 27 carbon atoms. The side chain ends with a carboxyl group, ionized at pH 7, that can be linked to the amino acid glycine or taurine.
In addition to the hydroxyl group at position 3, they have hydroxyl groups at positions 7 and/or 12.
All this makes them much more polar than cholesterol.
Since A and B rings are fused in cis configuration, the planar structure of the steroid nucleus is curved, and it is possible to identify:
- a concave side, which is hydrophilic because the hydroxyl groups and the carboxyl group of the side chain, with or without the linked amino acid, are oriented towards it;
- a convex side, which is hydrophobic because the methyl groups present at position 18 and 19 are orientated towards it.
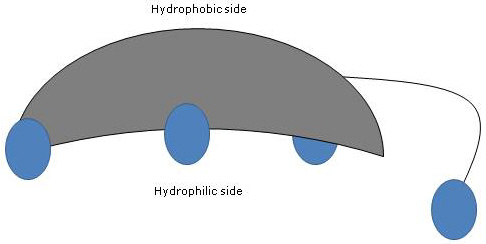
Therefore, having both polar and nonpolar groups, they are amphiphilic molecules and excellent surfactants. However, their chemical structure makes them different from many other surfactants, often composed of a polar head region and a nonpolar tail.
Primary, conjugated and secondary bile salts
Primary bile acids are those synthesized directly from cholesterol in the hepatocytes. In humans, the most important are cholic acid and chenodeoxycholic acid, which make up 80 percent of all bile acids.
Before being secreted into the biliary tree, they are almost completely conjugated, up to 98 percent, with the glycine or taurine, to form glycoconjugates and tauroconjugates, respectively. In particular, approximately 75 percent of cholic acid and chenodeoxycholic acid are conjugated with glycine, to form glycocholic acid and glycochenodeoxycholic acid, the remaining 25 percent with taurine, to form taurocholic acid and taurochenodeoxycholic.
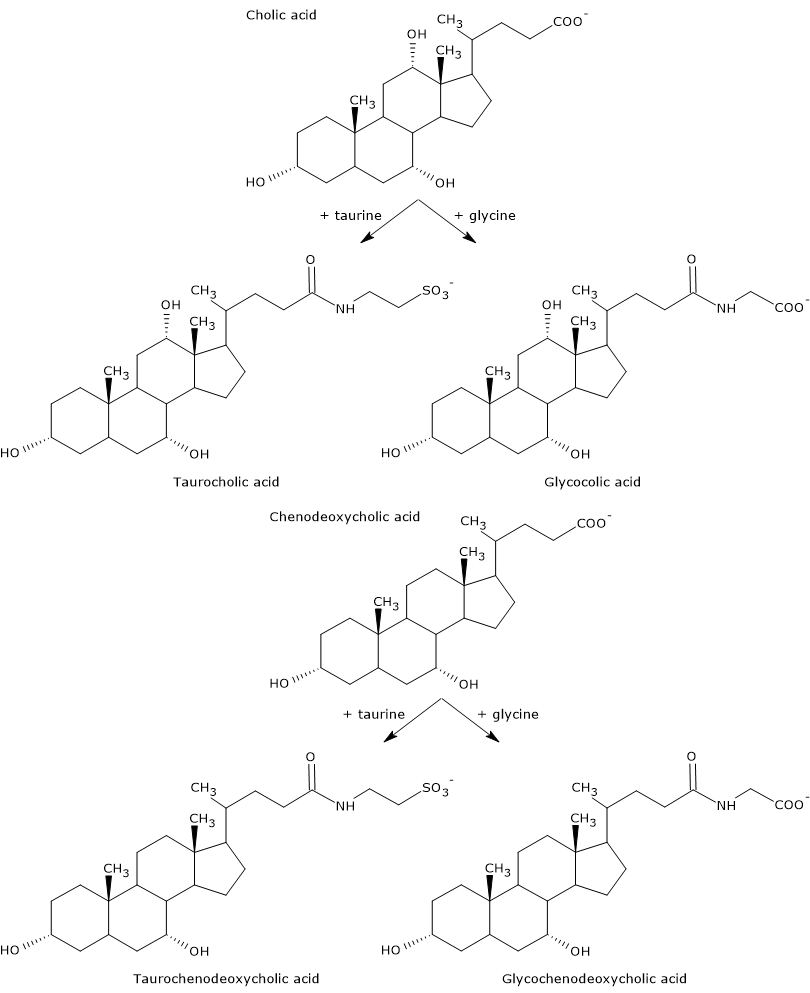
Conjugated bile acids are molecules with more hydrophilic groups than unconjugated bile acids, therefore they are much more effective emulsifiers. In fact, conjugation decreases the pKa of bile acids, from about 6, a value typical of non-conjugated molecules, to about 4 for glycocholic acid, and about 2 for taurocholic acid. This makes that conjugated bile acids are ionized in a broader range of pH to form the corresponding salts.
The hydrophilicity of the common acid and bile salts decreases in the following order: glycine-conjugated < taurine-conjugated < lithocholic acid < deoxycholic acid < chenodeoxycholic acid < cholic acid < ursodeoxycholic acid.
Finally, conjugation also decreases the cytotoxicity of primary bile acids.
Secondary bile acids are formed from primary bile acids which have not been reabsorbed from the small intestine. Once they reach the colon, they can undergo several modifications by bacteria of the gut microbiota, which is part of the larger human microbiota, to form secondary bile acids, which, along with short-chain fatty acids, are two major type of bacterial metabolites produced in the gut. They make up the remaining 20 percent of the body’s bile acid pool.
Another way of categorizing bile salts is based on their conjugation with glycine and taurine and their degree of hydroxylation. On this basis, three categories are identified.
- Trihydroxy conjugates, such as taurocholic acid and glycocholic acid.
- Dihydroxy conjugates, such as glycodeoxycholic acid, glycochenodeoxycholic acid, taurochenodeoxycholic acid, and taurodeoxycholic acid. They account for about 60 percent of bile salts present in the bile.
- Unconjugated forms, such as cholic acid, deoxycholic acid, chenodeoxycholic acid, and lithocholic acid.
Function of bile acids
All their physiological functions are performed in the conjugated form.
- They are the major route for the elimination of cholesterol from human body.
Indeed, humans do not have the enzymes to break open the cyclohexane rings or the cyclopentane ring of the steroid nucleus, nor to oxidize cholesterol to CO2 and water.
The other mechanism to eliminate the steroid from the body is as cholesterol per se in the bile. - Bile salts are strong surfactants. And in particular, di- and trihydroxy conjugates are the best surfactants among bile acids, much more effective than unconjugated counterparts, since they have more polar groups.
Once in contact with apolar lipids in the lumen of the small intestine, the convex apolar surface interacts with the apolar lipids, such as triglycerides, cholesterol esters, and ester of fat-soluble vitamins, whereas the concave polar surface interacts with the surrounding aqueous medium. This increases the dispersion of apolar lipids in the aqueous medium, as it allows the formation of tiny lipid droplets, increasing the surface area for lipase activity, mainly pancreatic lipase, (bile salts also play a direct role in the activation of this enzyme), andintestinal esterase activity. Subsequently, they facilitate lipid absorption, as well as absorption of fat soluble vitamins by the intestinal mucosa, thanks to the formation of mixed micelles.
Bile acids perform a similar function in the gallbladder where, forming mixed micelles with phospholipids, they prevent the precipitation of cholesterol.
Note: as a consequence of the arrangement of polar and nonpolar groups, bile acids form micelles in aqueous solution, usually made up of less than 10 monomers, as long as their concentration is above the so-called critical micellar concentration or CMC.
- At the intestinal level, they modulate the secretion of pancreatic enzymes and cholecystokinin.
- In the small and large intestine, they have a potent antimicrobial activity, mainly deoxycholic acid, in particular against Gram-positive bacteria. This activity may be due to oxidative DNA damage, and/or to the damage of the cell membrane. Therefore, they play an important role in the prevention of bacterial overgrowth, but also in the regulation of gut microbiota composition.
- In the last few years, it becomes apparent their regulatory role in the control of energy metabolism, and in particular for the hepatic glucose handling.
Enterohepatic circulation
After fat intake, enteroendocrine cells of the duodenum secrete cholecystokinin into the blood stream. Hormone binding to receptors on smooth muscle cells of the gallbladder promotes their contraction; the hormone also causes the relaxation of the sphincter of Oddi. All this results in the secretion of the bile, and therefore of bile acids into the duodenum.
Under physiological conditions, human bile salt pool is constant, and equal to about 3-5 g. This is made possible by two processes:
- their intestinal reabsorption;
- their de novo synthesis.
Up to 95 percent of the secreted bile salts is reabsorbed from the gut, not together with the products of lipid digestion, but through a process called enterohepatic circulation.
It is an extremely efficient recycling system, which seems to occur at least two times for each meal, and includes the liver, the biliary tree, the small intestine, the colon, and the portal circulation through which reabsorbed molecules return to the liver. Such recirculation is necessary since liver’s capacity to synthesize bile acids is limited and insufficient to satisfy intestinal needs if the bile salts were excreted in the feces in high amounts.
Most of the bile salts are reabsorbed into the distal ileum, the lower part of the small intestine, by a sodium-dependent transporter within the brush border of the enterocytes, called sodium-dependent bile acid transporter or ASBT, which carries out the cotransport of a molecule of bile acid and two sodium ions.
Within the enterocyte, it is thought that bile acids are transported across the cytosol to the basolateral membrane by the ileal bile acid-binding protein or IBABP. They cross the basolateral membrane by the organic solute transporter alpha-beta or OSTalpha/OSTbeta, pass into the portal circulation, and, bound to albumin, reach the liver.
It should be noted that a small percentage of bile acids reach the liver through the hepatic artery.
A hepatic level, their extraction is very efficient, with a first-pass extraction fraction ranging from 50 to 90 percent, a percentage that depends on bile acid structure. The uptake of conjugated bile acids is mainly mediated by a Na+-dependent active transport system, that is, the sodium-dependent taurocholate cotransporting polypeptide or NTCP. However, a sodium-independent uptake can also occur, carried out by proteins of the family of organic anion transporting polypeptides or OATP, mainly OATP1B1 and OATP1B3.
The rate limiting step in the enterohepatic circulation is their canalicular secretion, largely mediated by the bile salt export pump or BSEP, in an ATP-dependent process. This pump carries monoanionic bile salts, which are the most abundant. Bile acids conjugated with glucuronic acid or sulfate, which are dianionic, are transported by different carriers, such as MRP2 and BCRP.
Note: Serum levels of bile acids vary on the basis of the rate of their reabsorption, and therefore they are higher during meals, when the enterohepatic circulation is more active.
Intestinal metabolism
Bile acids which escape ileal absorption pass into the colon where they partly undergo modifications by intestinal microbiota and are converted to secondary bile acids.
The main reactions are listed below.
- Deconjugation
On the side chain, hydrolysis of the C24 N-acyl amide bond can occur, with release of unconjugated bile acids and glycine or taurine. This reaction is catalyzed by bacterial hydrolases present both in the small intestine and in the colon. - 7alpha-Dehydroxylation
Quantitatively, it is the most important reaction, carried out by colonic bacterial dehydratases that remove the hydroxyl group at position 7 to form 7-deoxy bile acids. In particular, deoxycholic acid is formed from cholic acid, and lithocholic acid, a toxic secondary bile acid, from chenodeoxycholic acid.
It should be noted that 7alpha-dehydroxylation, unlike oxidation and epimerization, can only occur on unconjugated bile acids, and therefore, deconjugation is an essential prerequisite. - Oxidation and epimerization
They are reactions involving the hydroxyl groups at positions 3, 7 and 12, catalyzed by bacterial hydroxysteroid dehydrogenases. For example, ursodeoxycholic acid derives from the epimerization of chenodeoxycholic acid.
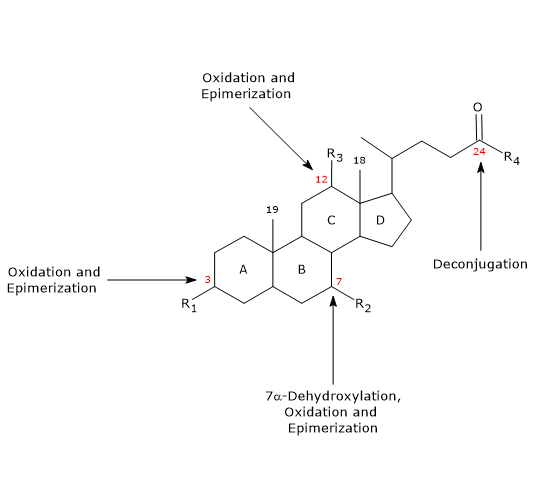
Some of the secondary bile acids are then reabsorbed from the colon and return to the liver. In the hepatocytes, they are reconjugated, if necessary, and resecreted. Those that are not reabsorbed, are excreted in the feces.
While oxidations and deconjugations are carried out by a broad spectrum of anaerobic bacteria, 7alpha-dehydroxylations is carried out by a limited number of colonic anaerobes.
7alpha-Dehydroxylations and deconjugations increase the pKa of the bile acids, and therefore their hydrophobicity, allowing a certain degree of passive absorption across the colonic wall.
The increase of hydrophobicity is also associated with an increased toxicity of these molecules. And a high concentration of secondary bile acids in the bile, blood, and feces has been associated to the pathogenesis of colon cancer.
Soluble fibers and reabsorption
The reabsorption of bile salts can be reduced by chelating action of soluble fibers, such as those found in fresh fruits, legumes, oats and oat bran, which bind them, decreasing their uptake. In turn, this increases bile acid de novo synthesis, up-regulating the expression of the 7alpha-hydroxylase and sterol 12alpha-hydroxylase, and thereby reduces hepatocyte cholesterol concentration.
The depletion of hepatic cholesterol increases the expression of the LDL receptor, and thus reduces plasma concentration of LDL cholesterol. On the other hand, it also stimulates the synthesis of HMG-CoA reductase, the key enzyme in cholesterol biosynthesis.
Note: Some anti-cholesterol drugs act by binding bile acids in the intestine, thereby preventing their reabsorption.
Synthesis
Quantitatively, bile acids are the major product of cholesterol metabolism.
As previously said, enterohepatic circulation and their de novo synthesis maintain a constant bile acid pool size. In particular, de novo synthesis allows the replacement of bile salts excreted in the faces, about 5-10 percent of the body pool, namely ~ 0.5 g/day.
Below, the synthesis of cholic acid and chenodeoxycholic acid, and their conjugation with the amino acids taurine and glycine, is described.
There are two main pathways for bile acid synthesis: the classical pathway and the alternative pathway. In addition, some other minor pathways will also be described.
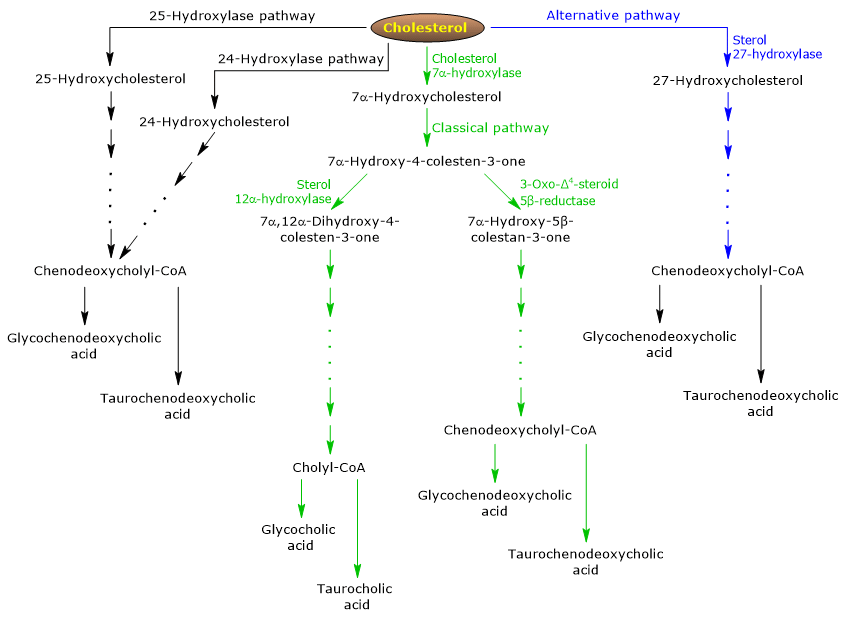
The classical or neutral pathway
In humans, up to 90 percent of bile salts are produced via the classical pathway, also referred to as “neutral” pathway since intermediates are neutral molecules.
It is a metabolic pathway present only in the liver, that consists of reactions catalyzed by enzymes localized in the cytosol, endoplasmic reticulum, peroxisomes, and mitochondria, and whose end products are the conjugates of cholic acid and chenodeoxycholic acid.
- The first reaction is the hydroxylation at position 7 of cholesterol, to form 7alpha-hydroxycholesterol. The reaction is catalyzed by cholesterol 7alpha-hydroxylase or CYP7A1 (E.C. 1.14.14.23). It is an enzyme localized in the endoplasmic reticulum, and catalyzes the rate-limiting step of the pathway.
Cholesterol + NADPH + H+ + O2 → 7alpha-Hydroxycholesterol + NADP+ + H2O
- 7alpha-Hydroxycholesterol undergoes oxidation of the 3beta-hydroxyl group and the shift of the double bond from the 5,6 position to the 4,5 position, to form 7alpha-hydroxy-4-cholesten-3-one. The reaction is catalyzed by 3beta-hydroxy-Δ5-C27-steroid oxidoreductase or HSD3B7 (E.C. 1.1.1.181), an enzyme localized in the endoplasmic reticulum.
- 7alpha-Hydroxy-4-cholesten-3-one can follow two routes:
to enter the pathway that leads to the synthesis of cholic acid, through the reaction catalyzed by 7alpha-hydroxy-4-cholesten-3-one12alpha-monooxygenase or sterol 12alpha-hydroxylase or CYP8B1 (E.C. 1.14.18.8), an enzyme localized in the endoplasmic reticulum;
to enter the pathway that leads the synthesis of chenodeoxycholic acid, through the reaction catalyzed by 3-oxo-Δ4-steroid 5beta-reductase or AKR1D1 (E.C. 1.3.1.3), a cytosolic enzyme.
It should be underlined that the activity of sterol 12alpha-hydroxylase determines the ratio of cholic acid to chenodeoxycholic acid, and, ultimately, the detergent capacity of bile acid pool. And in fact, the regulation of sterol 12alpha-hydroxylase gene transcription is one of the main regulatory step of the classical pathway.
Therefore, if 7alpha-hydroxy-4-cholesten-3-one proceeds via the reaction catalyzed by sterol 12alpha-hydroxylase, the following reactions will occur.
- 7alpha-Hydroxy-4-cholesten-3-one is hydroxylated at position 12 by sterol 12alpha-hydroxylase, to form 7alpha,12alpha-dihydroxy-4-cholesten-3-one.
- 7alpha,12alpha-Dihydroxy-4-cholesten-3-one undergoes reduction of the double bond at 4,5 position, in the reaction catalyzed by 3-oxo-Δ4-steroid5beta-reductase, to form 5beta-cholestan-7alpha,12alpha-diol-3-one.
- 5beta-Cholestan-7alpha,12alpha-diol-3-one undergoes reduction of the hydroxyl group at position 4, in the reaction catalyzed by 3 alpha-hydroxysteroid dehydrogenase or AKR1C4 (EC 1.1.1.213), a cytosolic enzyme, to form 5beta-cholestan-3 alpha,7alpha,12alpha-triol.
- 5beta-Cholestan-3alpha,7alpha,12alpha-triol undergoes oxidation of the side chain via three reactions catalyzed by sterol 27-hydroxylase or CYP27A1 (EC 1.14.15.15). It is a mitochondrial enzyme also present in extrahepatic tissues and macrophages, which introduces a hydroxyl group at position 27. The hydroxyl group is oxidized to aldehyde, and then to carboxylic acid, to form 3 alpha,7 alpha,12 alpha-trihydroxy-5 beta-cholestanoic acid.
- 3alpha,7alpha,12alpha-Trihydroxy-5beta-cholestanoic acid is activated to its coenzyme A ester, 3alpha,7alpha,12alpha-trihydroxy-5beta-cholestanoyl-CoA, in the reaction catalyzed by either very long chain acyl-CoA synthetase or VLCS (EC 6.2.1.-), or bile acid CoA synthetase or BACS (EC 6.2.1.7), both localized in the endoplasmic reticulum.
- 3alpha,7alpha,12alpha-Trihydroxy-5beta-cholestanoyl-CoA is transported to peroxisomes where it undergoes five successive reactions, each catalyzed by a different enzyme. In the last two reactions, the side chain is shortened to four carbon atoms, and finally cholylCoA is formed.
- In the last step, the conjugation, via amide bond, of the carboxylic acid group of the side chain with the amino acid glycine or taurine occurs. The reaction is catalyzed by bile acid-CoA:amino acid N-acyltransferase or the BAAT (EC 2.3.1.65), which is predominantly localized in peroxisomes.
The reaction products are thus the conjugated bile acids: glycocholic acid and taurocholic acid.
If 7alpha-hydroxy-4-cholesten-3-one does not proceed via the reaction catalyzed by sterol 12alpha-hydroxylase, it enters the pathway that leads to the synthesis of chenodeoxycholic acid conjugates, through the reactions described below.
- 7alpha-Hydroxy-4-cholesten-3-one is converted to 7alpha-hydroxy-5beta-cholestan-3-one in the reaction catalyzed by 3-oxo-Δ4-steroid 5 beta-reductase.
- 7alpha-Hydroxy-5beta-cholestan-3-one is converted to 5beta-cholestan-3alpha,7alpha-diol in the reaction catalyzed by 3alpha-hydroxysteroid dehydrogenase.
Then, the conjugated bile acids glycochenodeoxycholic acid and taurochenodeoxycholic acid are formed by modifications similar to those seen for the conjugation of cholic acid, and catalyzed mostly by the same enzymes.
Note: Unconjugated bile acids formed in the intestine must reach the liver to be reconjugated.
The alternative or acidic pathway
It is prevalent in the fetus and neonate, whereas in adults it leads to the synthesis of less than 10 percent of the bile salts.
This pathway differs from the classical pathway in that:
- the intermediate products are acidic molecules, from which the alternative name “acidic pathway”;
- the oxidation of the side chain is followed by modifications of the steroid nucleus, and not vice versa;
- the final products are conjugates of chenodeoxycholic acid.
The first step involves the conversion of cholesterol into 27-hydroxycholesterol in the reaction catalyzed by sterol 27-hydroxylase.
27-Hydroxycholesterol can follow two routes.
Route A
- 27-hydroxycholesterol is converted to 3beta-hydroxy-5-cholestenoic acid in a reaction catalyzed by sterol 27-hydroxylase.
- 3beta-Hydroxy-5-cholestenoic acid is hydroxylated at position 7 in the reaction catalyzed by oxysterol 7alpha-hydroxylase or CYP7B1 (EC 1.14.13.100), an enzyme localized in the endoplasmic reticulum, to form 3beta-7alpha-dihydroxy-5-colestenoic acid.
- 3beta-7alpha-Dihydroxy-5-cholestenoic acid is converted to 3-oxo-7alpha-hydroxy-4-cholestenoic acid, in the reaction catalyzed by 3beta-hydroxy-Δ5-C27-steroid oxidoreductase.
- 3-Oxo-7alpha-hydroxy-4-cholestenoic acid, as a result of side chain modifications, forms chenodeoxycholic acid, and then its conjugates.
Route B
- 27-Hydroxycholesterol is converted to 7alpha,27-dihydroxycholesterol in the reaction catalyzed by oxysterol 7alpha-hydroxylase and cholesterol 7alpha-hydroxylase.
- 7alpha,27-Dihydroxycholesterol is converted to 7alpha,26-dihydroxy-4-cholesten-3-one in the reaction catalyzed by 3beta-hydroxy-Δ5-C27-steroid oxidoreductase;
7alpha, 26-Dihydroxy-4-cholesten-3-one can be transformed directly to conjugates of chenodeoxycholic acid, or can be converted to 3-oxo-7alpha-hydroxy-4-colestenoic acid, and then undergo side chain modifications and other reactions that lead to the synthesis of the conjugates of chenodeoxycholic acid.
Minor pathways
There are also minor pathways that contribute to bile salt synthesis, although to a lesser extent than classical and alternative pathways.
For example:
- A cholesterol 25-hydroxylase (EC 1.14.99.38) is expressed in the liver.
- A cholesterol 24-hydroxylase or CYP46A1 (EC 1.14.14.25) is expressed in the brain, and therefore, although the organ cannot export cholesterol, it exports oxysterols.
- A nonspecific 7alpha-hydroxylase has also been discovered. It is expressed in all tissues and appears to be involved in the generation of oxysterols, which may be transported to hepatocytes to be converted to chenodeoxycholic acid.
Additionally, sterol 27-hydroxylase is expressed in various tissues, and therefore its reaction products must be transported to the liver to be converted to bile salts.
Bile salts: regulation of synthesis
Regulation of bile acid synthesis occurs via a negative feedback mechanism, particularly on the expression of cholesterol 7alpha-hydroxylase and sterol 12alpha-hydroxylase.
When an excess of bile acids, both free and conjugated, occurs, these molecules bind to the nuclear receptor farnesoid X receptor or FRX, activating it: the most efficacious bile acid is chenodeoxycholic acid, while others, such as ursodeoxycholic acid, do not activate it.
FRX induces the expression of the transcriptional repressor small heterodimer partner or SHP, which in turn interacts with other transcription factors, such as liver receptor homolog-1 or LRH-1, and hepatocyte nuclear factor-4alpha or HNF-4alpha. These transcription factors bind to a sequence in the promoter region of 7alpha-hydroxylase and 12alpha-hydroxylase genes, region called bile acid response elements or BAREs, inhibiting their transcription.
One of the reasons why bile salt synthesis is tightly regulated is because many of their metabolites are toxic.
References
- Chiang J.Y.L. Bile acids: regulation of synthesis. J Lipid Res 2009;50(10):1955-1966. doi:10.1194/jlr.R900010-JLR200
- Gropper S.S., Smith J.L. Advanced nutrition and human metabolism. 6th Edition. Cengage Learning, 2012
- Moghimipour E., Ameri A., and Handali S. Absorption-enhancing effects of bile salts. Molecules 2015;20(8); 14451-14473. doi:10.3390/molecules200814451
- Monte M.J., Marin J.J.G., Antelo A., Vazquez-Tato J. Bile acids: Chemistry, physiology, and pathophysiology. World J Gastroenterol 2009;15(7):804-816. doi:10.3748/wjg.15.804
- Rosenthal M.D., Glew R.H. Medical biochemistry – Human metabolism in health and disease. John Wiley J. & Sons, Inc., Publication, 2009
- Sundaram S.S., Bove K.E., Lovell M.A. and Sokol R.J. Mechanisms of Disease: inborn errors of bile acid synthesis. Nat Clin Pract Gastroenterol Hepatol 2008;5(8):456-468. doi:10.1038/ncpgasthep1179